Learn about the steps and materials needed to make a nuclear weapon, a description of weapon designs, and a history of nuclear weapon tests.
Table of Contents |
---|
1. Implosion design |
2. Gun assembly design |
3. Thermonuclear weapons |
4. Nuclear weapon materials |
5. Effects of nuclear weapons |
Table 1 - Effective lethal radii for nuclear weapons |
Table 2 - Five psi radii for various yield nuclear weapons |
6. The first bombs and recent tests |
1. Implosion design
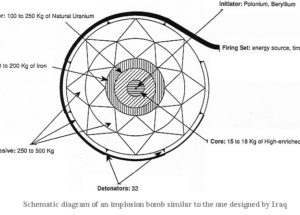
1.1 Legend for Figure 1
Firing Set: a wiring system that sends a large electrical impulse to set off the detonators.
Detonators: devices used to ignite the high explosive section of the weapon.
High explosive: shaped charges made from materials such as HMX, RDX, and TATB.
Tamper: a dense metal such as natural uranium that holds the core together by inertia.
Reflector: a material such as beryllium that bounces neutrons back into the core to increase fission.
Core: made of metallic plutonium-239 or uranium-235, the most widely used “fissile” isotopes, so-called because of their natural property to split, or fission, when struck by a low energy (or “thermal”) neutron.
Initiator: a source of neutrons that can be a pellet (made of a sandwich of polonium-210 and beryllium separated by a layer of gold foil) placed in the center of the core, or that can be a tubular device mounted on the outside of the bomb that shoots a burst of neutrons into the core at the moment of detonation.
1.2 Mechanics of an implosion device
In a so-called “implosion” weapon, which is the most common design used today, the weapon is armed with detonators that initiate the explosion. Regardless of the way the weapon is delivered (missile, bomb, artillery shell) the detonators fire simultaneously to set off a charge of high explosives that ring the outer surface of the tamper. These high explosives are finely machined in a lens configuration that sends shock waves into the center of the weapon. The shock waves compress the fissile core of uranium or plutonium into what is known as a supercritical state.
The physical basis of a nuclear weapon lies in creating this supercritical state. When a fissile nucleus is struck by a neutron, the nucleus splits and emits additional neutrons and a large amount of energy. These newly freed neutrons can then strike and fission other nuclei, which produces a chain reaction. When the fissile material is arranged in such a manner that the fission of one nucleus leads to the fission of one other nucleus, the chain reaction is self-sustaining and the material is said to have reached its critical mass. Thus, supercriticality is when the fission of one nucleus in the chain reaction leads to the fission of more than one other nucleus.
Each fission event releases a large amount of energy in the form of light, heat, and radiation, so successive generations of fission events in the chain reaction will produce exponentially increasing amounts of energy. The key is to create and sustain a chain reaction long enough to produce the desired explosive energy before the fissile core rips itself apart due to the internal pressure created by the energy release. For example, 99.9% of the energy released in a 100 kiloton (1 kiloton = 1,000 tons of TNT) nuclear explosion is released in the last 7 generations, out of a total of over 50 generations, and occurs in approximately 0.07 microseconds.
The purpose of the tamper is to hold the core together long enough to allow the necessary fission generations to occur, otherwise the weapon will “fizzle” and not release the expected energy yield. The fission taking place in the core exerts pressure on the tamper, which responds by pushing back on the core by virtue of the tamper’s inertia. The initiator and reflector also act to prevent fizzling and increase the yield. The polonium in the “pellet” type initiator releases alpha particles, a form of radiation, that are blocked by gold foil until the foil is broken by the implosive shockwave. The alpha particles then hit the beryllium and produce a reaction that releases neutrons. Thus, the initiator provides a burst of neutrons to quickly start the chain reaction and maximize fission. The reflector is used to bounce neutrons produced by fission back into the core to fission additional nuclei and increase the yield.
In a variation of this implosion design, a “boosted” yield can be achieved by injecting deuterium and tritium gas into the center of the fissile core. Deuterium occurs in nature; tritium is produced by irradiating lithium in a reactor. The heat and pressure created during the fissioning of the core cause a fusion reaction to occur in the gas, which then releases more neutrons. The extra neutrons act to fission more of the fissile core and increase the yield. Boosting can multiply the yield by a factor of 10.
When properly put together, an implosion weapon can produce an explosion on the order of a few kilotons to hundreds of kilotons.
2. Gun assembly design
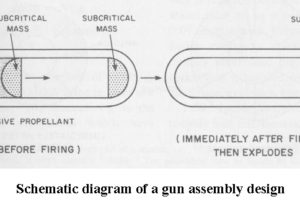
2.1 Legend for Figure 2
Explosive propellant: chemical explosive, analogous to but not the same as the high explosive in an implosion design.
Tamper: not shown in the diagram but used for the same purpose and composed of the same material as in an implosion design.
Subcritical mass and supercritical mass: exclusively uranium-235 for this design; plutonium-239 will not work.
2.2 Mechanics
The basic physics of this design is similar to that of the implosion design. Both weapons assemble a supercritical mass of fissile material and use a tamper to hold the core together long enough to produce the desired nuclear explosion. However, the mechanics of a gun design are much simpler, which means that the device is much easier to make.
The uranium-235 is machined into two sub-critical masses, which if joined together would be greater than a critical mass. Then, one of the sub-critical masses is placed at one end of a tube in front of a propellant, and the other is placed at the other end of the tube. When the propellant is detonated, it shoots the first mass down the tube at a high speed. When this mass collides with the second they create a supercritical mass, which produces a fission chain reaction. Once again, the tamper acts to hold the fissile core together long enough to prevent the weapon from fizzling. A neutron generator can also be placed at the core’s center to induce greater fission.
Compared to an implosion weapon, the gun assembly acts slower, is not as powerful, and uses far more fissile material. However, the explosive power is still in the range of tens of kilotons.
3. Thermonuclear weapons
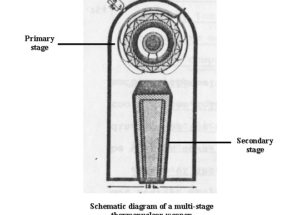
3.1 Legend for Figure 3
Primary stage: fission implosion device as described in section 1, typically boosted with deuterium-tritium gas.
Secondary stage: a fusion fuel charge composed of lithium deuteride, which contains at its center a cylindrical rod of uranium-235 or plutonium-239, and is surrounded by a casing of uranium metal. The fusion reaction commonly employed is that of deuterium and tritium. The tritium is created when the lithium in the lithium deuteride reacts with a neutron.
3.2 Mechanics
A thermonuclear weapon employs fusion as well as fission. Fusion is the bringing together of two nuclei to form a new nucleus. The most common fusion reaction is that of two isotopes of hydrogen, namely tritium and deuterium, thus the term “hydrogen bomb.” These isotopes combine to form helium-4 and a neutron. Similar to fission, the goal is to create a self-sustaining chain reaction that releases exponentially increasing amounts of energy.
Fusion is not limited by the requirement of a critical mass, so these weapons can reach theoretically limitless power. Often they are on the order of a few megatons (1 megaton = 1,000,000 tons of TNT). The largest nuclear weapon ever detonated was an approximately 59 megaton thermonuclear bomb produced by the Soviet Union. Fusion, however, requires higher temperatures and densities than can be achieved by chemical high explosives, so a nuclear fission explosion is used to create the necessary temperature and density. The result is a two-stage reaction in which a fission bomb explodes first and sets off the secondary, fusion part of the weapon. As can be concluded from this discussion, thermonuclear weapons are not a primary proliferation concern because fission weapon technology must first be mastered before a thermonuclear weapon can be developed.
A multi-stage thermonuclear weapon is called a Teller-Ulam configuration. The primary stage has the same basic design as an implosion fission weapon, described in section 1. After the primary stage is detonated, the x-rays it releases cause the pressure and temperature inside the weapon casing to reach the conditions necessary to achieve a thermonuclear reaction in the fusion fuel. The yield of the fusion fuel is increased when the fissile rod in its center reaches a supercritical state and begins itself to fission. As the fusion fuel reacts, it releases high-energy neutrons that also fission the uranium-238 nuclei that are in the uranium metal casing wrapped around the fusion fuel. In a typical configuration, fission and fusion each contribute about half the overall energy yield.
3.3 Enhanced radiation (neutron) weapons
Another class of thermonuclear weapons creates the maximum amount of radiation possible while minimizing the effects caused by blast. These are called enhanced radiation, or neutron bombs. They rely on fusion between deuterium and tritium to produce a lethal radius of neutrons and gamma rays. The goal is to produce a low yield weapon (deliverable by an artillery shell, for example) that inflicts prompt casualties on troops by radiation but leaves intact structures that otherwise would be destroyed by blast effects.
Because fusion releases many times more neutrons than fission for a given weight of fuel, a neutron bomb can create a larger radius inside which there is a lethal dose of nuclear radiation than a small fission bomb can. A one kiloton neutron bomb, for example, creates about the same lethal radius of nuclear radiation as a 10 kiloton fission weapon. This means that by using a neutron bomb, it is possible to achieve a given radius of lethality with only one tenth of the blast damage that would otherwise be required. These are tactical, not strategic weapons because of their small size. When detonated in the air, they have the additional advantage of producing little residual radiation (fallout) so it is plausible to think of them as battlefield weapons.
4. Nuclear weapon materials
Both plutonium-239 and uranium-235 have been used as nuclear explosives in fission weapons. Approximately ninety percent of the effort that went into making America’s first bombs was devoted to producing these two materials, which is no easy task.
4.1 Plutonium
The world’s first nuclear explosion was achieved with plutonium, a man-made element produced in nuclear reactors. Plutonium is created when an atom of uranium-238 absorbs a neutron and becomes plutonium-239. The reactor generates the neutrons in a controlled chain reaction. For the neutrons to be absorbed by the uranium their speed must be slowed by passing them through a substance known as a “moderator.” Graphite and heavy water have been used as moderators in reactors fueled by natural uranium. For graphite to succeed as a moderator it must be exceptionally pure; impurities will halt the chain reaction. Heavy water looks and tastes like ordinary water but contains atoms of deuterium instead of atoms of hydrogen. For heavy water to succeed as a moderator, it too must be pure; it must be free of significant contamination by ordinary water, with which it is mixed in nature.
4.1.1 Plutonium needed to make a weapon
- 4 kilograms: weight of a solid sphere of plutonium just large enough to achieve a critical mass with a beryllium reflector. Diameter of such a sphere: 2.86 in (7.28 cm). Diameter of a regulation baseball: 2.90 in (7.36 cm).
- 4.4 kilograms: estimated amount used in Israel’s fission bombs.
- 5 kilograms: estimated amount needed to manufacture a first-generation fission bomb today.
- 6.1 kilograms: amount used in the “Trinity” test in 1945 and in the bomb dropped on Nagasaki.
- 15 kilograms: weight of a solid sphere of plutonium just large enough to achieve a critical mass without a reflector. Diameter of such a sphere: 4.44 in (11.3 cm). Diameter of a regulation softball: 3.82 in (9.7 cm).
4.1.2 Plutonium generated by various reactors
- 5.5 to 8 kilograms/year: North Korea’s 20-30 megawatt (thermal) Yongbyon reactor moderated by graphite.
- 9 kilograms/year: India’s 40 megawatt (thermal) Cirus reactor moderated by heavy water.
- 12 kilograms/year: Pakistan’s 50 megawatt (thermal) Khushab reactor moderated by heavy water.
- 25 kilograms/year: India’s 100 megawatt (thermal) Dhruva reactor moderated by heavy water.
- 40 kilograms/year: Israel’s more than 100 megawatt (thermal) Dimona reactor moderated by heavy water.
- 230 kilograms/year: Iran’s 1,000 megawatt (electric) Bushehr reactor being supplied by Russia and moderated by ordinary (light) water (not yet in operation).
4.1.3 Heavy water needed for a small reactor to make nuclear weapons:
- 19 metric tons: India’s 40 megawatt (thermal) Cirus reactor.
- More than 36 metric tons: Israel’s more than 100 megawatt (thermal) Dimona reactor.
- 78 metric tons: India’s 100 megawatt (thermal) Dhruva reactor.
4.1.4 Chemical extraction of plutonium
Before being used in a bomb, plutonium must be separated from the intensely hot, and highly radioactive fuel rods in which it is created in a reactor. To achieve this separation, a specially shielded chemical plant is needed to chop the fuel rods into pieces, dissolve the radioactive spent fuel in acid, and then extract the plutonium in pure form.
4.1.5 Some of the equipment needed to make plutonium
- A nuclear reactor and its associated equipment, such as heavy water or graphite.
- A plant for fabricating fresh nuclear reactor fuel.
- A plant for chemically extracting plutonium from spent reactor fuel.
- Large quantities of pure reagents for PUREX or other plutonium extraction processes.
- Remote handling equipment to process irradiated fuel.
- Shielded casks to transport radioactive material.
- High-density/lead glass radiation shielding windows.
- Radiation-hardened TV cameras.
- Fuel chopping machines.
4.2 Uranium-235
The world’s second nuclear explosion was achieved with uranium-235. This isotope, like plutonium, is unstable and fissions when struck by a neutron. It is, however, found in natural uranium at a concentration of only 0.7 percent. To be useful in a nuclear weapon, the concentration must be increased. This is accomplished by a process known as enrichment. Because the isotopes of uranium are identical chemically, the enrichment process exploits the slight difference in their masses. Uranium enriched to greater than twenty percent uranium-235 is called highly enriched. Nuclear weapons typically use a concentration of more than 90 percent uranium-235.
4.2.1 Uranium-235 needed to make a weapon
- 15 kilograms: weight of a solid sphere of 100 percent uranium-235 just large enough to achieve a critical mass with a beryllium reflector. Diameter of such a sphere: 4.48 in (11.4 cm). Diameter of a regulation softball: 3.82 in (9.7 cm).
- 16 kilograms: amount needed for an Iraqi bomb design found by UN inspectors.
- 50 kilograms: weight of a solid sphere of 100 percent uranium-235 just large enough to achieve a critical mass without a reflector. Diameter of such a sphere: 6.74 in (17.2 cm), comparable to an average “honeydew” melon.
- 60 kilograms: reported amount used in Hiroshima bomb “Little Boy.”
4.2.2 Various methods used to enrich uranium
- Electromagnetic Isotope Separation (EMIS)
In this process, uranium atoms are ionized (given an electrical charge) then sent in a stream past powerful magnets. The heavier uranium-238 atoms are deflected less in their trajectory than the lighter uranium-235 atoms by the magnetic field, so the isotopes separate and can be captured by collectors. The process is repeated until a high concentration of uranium-235 is achieved. An American version of the EMIS process, featuring “calutrons,” was used in the Manhattan Project. EMIS was also used in Iraq’s illicit effort to enrich uranium. - Gaseous Diffusion
In this process, gaseous uranium hexafluoride (UF6) flows through a porous membrane of nickel or aluminum oxide. Lighter molecules of uranium-235 within the UF6 diffuse through the porous barrier at a faster rate than the heavier molecules of uranium-238. Because the difference in velocities between the two isotopes is small, the process must be repeated thousands of times to achieve weapon-usable uranium-235. The United States used this enrichment method to fuel its early fission bombs, and China used it to build its first nuclear weapons in the 1950s and 1960s. - Gas Centrifuge
Gaseous UF6 is fed into a cylindrical rotor that spins at a high speed inside an evacuated casing. Centrifugal forces cause the heavier uranium-238 to move closer to the outer wall than the lighter uranium-235, thus partially separating the isotopes. This separation is increased by a relatively slow axial countercurrent flow of gas within the centrifuge that concentrates enriched gas at one end and depleted gas at the other. Numerous repetitions of the process, employing thousands of centrifuges in arrangements called cascades, are needed to concentrate the uranium-235 to weapon-grade. Pakistan used gas centrifuges to enrich the uranium for its first nuclear weapon, and both Iraq and Iran have built gas centrifuges in illicit programs hidden from international inspectors. - Aerodynamic Processes
In the Becker nozzle process a mixture of gaseous UF6 and helium is compressed and then directed along a curved wall at high speed. The heavier uranium-238-bearing molecules move preferentially out to the wall relative to those containing uranium-235. At the end of the deflection, the gas jet is split by a knife edge into a light fraction and a heavy fraction, which are withdrawn separately. - Atomic Vapor Laser Isotope Separation (AVLIS)
The AVLIS process uses dye lasers tuned so that only the uranium-235 atoms absorb the laser light. As the uranium-235 atom absorbs the light, its electrons are excited to a higher energy state. When enough energy is absorbed, a uranium-235 atom will eject an electron and become a positively charged ion. The uranium-235 ions may then be deflected by an electrostatic field to a product collector. The uranium-238 atoms remain neutral and are not collected. - Molecular Laser Isotope Separation (MLIS)
The MLIS separation process consists of two steps. In the first, UF6 is excited by an infrared laser system, which selectively excites the molecules bearing uranium-235, leaving the molecules bearing uranium-238 unexcited. In the second step, photons from a second laser system (infrared or ultraviolet) preferentially dissociate the excited uranium-235 to form uranium pentafluoride molecules bearing uranium-235 and free fluorine atoms. The uranium-235 then precipitates as a powder that can be filtered from the gas stream. - Thermal Diffusion
Thermal diffusion uses the transfer of heat across a thin liquid or gas to accomplish isotope separation. By cooling a vertical film on one side and heating it on the other, the resultant convection currents will produce an upward flow along the hot surface and a downward flow along the cold surface. Under these conditions, the lighter uranium-235 molecules will diffuse toward the cold surface. These two diffusive motions combined with the convection currents will cause the lighter uranium-235 molecules to concentrate at the top of the film and the heavier uranium-238 molecules to concentrate at the bottom of the film.
4.2.3 Critical equipment needed to enrich uranium
Most of the enrichment processes also require that the natural uranium be converted into a gaseous form prior to enrichment, typically uranium hexafluoride (UF6). Thus, a separate chemical processing plant must be constructed to convert the uranium into gaseous form.
Critical items:
- High-strength aluminum, maraging steel, or graphite to make centrifuge rotors.
- Spin forming or flow forming machines.
- Filament winding machines.
- Balancing machines.
- Sintered nickel for gaseous diffusion barriers.
- High-purity fluorine.
- Special valves, seals and pipe lining material for handling UF6.
- Pumps for moving uranium hexafluoride at high pressure.
- Laser isotope separation equipment.
4.3 Weapon design and production
In addition to the plutonium or highly enriched uranium needed to fuel a weapon, other components are required to achieve a successful detonation. These typically require high-precision manufacturing, which can be accomplished only with specialized equipment or materials. Such components also require specialized testing equipment. Selected components and equipment are listed below.
- Firing sets: containing high-energy, low impedance capacitor banks, and high current, high-speed switches (thyratrons, krytrons, sprytrons).
- High explosives: substances or mixtures known as HMX, RDX, TATB, HNS.
- High-speed recording devices (oscilloscopes, streak cameras) and high-speed photography, flash x-rays and mechanical-electronic diagnostics such as pin-domes.
- Reflector material, such as beryllium and its alloys.
- Vacuum furnaces for casting uranium and plutonium.
- Neutron generators.
5. Effects of nuclear weapons
The energy released by a nuclear explosion comes in several forms: pressure from the blast, thermal radiation, nuclear radiation, and an electromagnetic pulse. The damage inflicted by the various effects depends upon the size and type of the explosion.
5.1 Blast
A large portion of the damage caused by a nuclear weapon is from the blast. The detonation produces a drastic increase in atmospheric pressure and severe transient winds. Due to the extreme temperature and pressure created, a massive shock wave is promulgated outward from the detonation point. The high “overpressure” destroys buildings, and the wind causes fatal collisions between people and nearby objects.
5.2 Thermal radiation
The intense heat from a nuclear explosion causes burns to human skin and a temporary condition called “flashblindness.” The maximum temperature achieved by a fission weapon is several tens of million degrees. A standard chemical high-explosive produces only 5,000 degrees centigrade (9,000 degrees Fahrenheit). A one megaton explosion can produce third degree burns (which destroy skin tissue) at a distance of five miles. The extent to which burns are inflicted depends on weather conditions. The heat from the explosion can also ignite fires and under some conditions can produce a “firestorm.” Thermal effects predominate in weapons in the megaton range.
5.3 Nuclear radiation
The nuclear radiation resulting from a nuclear explosion can be divided into two categories: initial and residual. The initial radiation consists of neutrons and gamma rays, which can travel great distances, penetrate considerable thicknesses of material, and inflict fatal damage on human tissue. Initial radiation can be intense but has a limited range. For large nuclear weapons, the range of initial radiation is less than the range of lethal blast and thermal effects. For small weapons, direct radiation may be the lethal effect with the greatest range.
While there is some controversy about the effect of nuclear radiation on the human body, it is estimated that an exposure of 600 rem or greater within one week will result in a 90% chance of death within a few weeks. For a one kiloton blast, initial radiation levels of at least 600 rem extend out 0.8 km from the blast. For a one megaton surface blast, the 600 rem exposure radius would be about 2.7 km.
Residual radiation is often termed fallout, and it can affect both the immediate blast area and areas farther away. Fallout is caused by particles that are scooped up when the nuclear fireball touches the earth. If the nuclear burst is high in the air, fallout is minimal. The scooped-up particles can be carried some distance by the wind before falling back to earth, and their concentration in any one location depends on local weather conditions. Fallout can cause severe contamination to soil, vegetation and groundwater. A steady northwest wind, for example, blowing across a one megaton ground burst in Detroit, could carry enough residual radiation to inflict acute radiation sickness to exposed persons in Cleveland. The residual radiation decays over time, by a factor of ten after seven hours, a factor of 100 after 49 hours and a factor of 1,000 after two weeks. Depending on the conditions of the blast, radiation levels can persist above permissible peace time levels for months or years in areas around the explosion.
5.4 Overall effects
Taking into account the effects of the total energy released in a nuclear explosion, Table 1 summarizes the effective lethal radii for weapons of various yields. The effective lethal radius is defined as the radius at which the fatality rate is approximately 50% in a typical urban area. This can also be estimated as a ring inside which the mean lethal overpressure is approximately five pounds per square inch. This is the amount of pressure needed to collapse a typical residence.
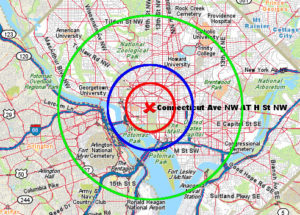
5.5 A Bomb over Washington, D.C.
Imagine that a nuclear weapon is detonated in Washington, D.C. at an optimum burst height over the intersection of Connecticut Ave N.W. and H Street N.W., which forms the northwest corner of Lafayette Park and lies approximately two blocks north of the White House. Using the definition of lethal radius as the area inside which the mean overpressure is five pounds per square inch, the lethal radius for such an event with weapons of various yields can be calculated. Table 2 and the map figure display the five pounds per square inch radii for weapons with yields of one kiloton, 20 kiloton, 100 kiloton and one megaton.
The radii given in Table 2 assume that the weapon detonates in air at the optimum height for creating damage. The bombs dropped on Hiroshima and Nagasaki, Japan, for example, exploded at a height of approximately 1,650 feet. Buildings in Washington, DC are limited to 160 feet. A weapon delivered in a vehicle, such as a cargo van, would not detonate at the optimum height. Instead, it would be a “surface burst.” For such an explosion the five psi radii given in Table 2 would be approximately 35% smaller. A surface burst would, on the other hand, create “fallout.” This consists of particles of soil and debris propelled into the air by the fireball, which would occur at ground level. An air burst creates little fallout because the fireball does not touch the ground.
6. The first bombs
United States
“Trinity”: World’s first nuclear explosion: July 16, 1945.
Location: Near Alamogordo, New Mexico.
Yield: 21 kilotons.
Fissile material used: Plutonium-239.
Amount: 6.1 kilograms.
Method of detonation: Implosion.
Amount of high-explosive wrapped around plutonium core: 2268 kilograms.
Method of production: Nuclear reactor at the Hanford Reservation.
“Little Boy”: First use of nuclear weapon in war: August 6, 1945.
Location: Hiroshima, Japan.
Detonation height: approximately 1,650 feet.
Delivery mechanism: Airdropped from B-29 bomber named Enola Gay.
Yield: 12.5 kilotons.
Fissile material used: Uranium-235.
Method of detonation: “Gun-type” device.
Method of production: “Calutron” electromagnetic isotope separation.
“Fat Man”: Second use of a nuclear weapon in war: August 9, 1945.
Location: Nagasaki, Japan.
Detonation Height: approximately 1,650 feet.
Delivery mechanism: Airdropped from B-29 bomber named Bockscar.
Yield: 22 kilotons.
Fissile material used: Plutonium-239.
Method of Detonation: Implosion.
Amount used: 6.2 kilograms.
“Ivy Mike”: First hydrogen bomb tested: November 1, 1952.
Location: Elugelab Island, Enewetak Atoll.
Yield: 10.4 megatons.
Soviet Union
“Joe 1”: First nuclear test: August 29, 1949.
Location: Semipalatinsk, Kazakhstan.
Yield: 10-20 kilotons.
Fissile material used: Plutonium-239.
Method of detonation: Implosion.
Method of production: Reactor.
“Joe 4”: First thermonuclear test: August 12, 1953.
Location: Possibly in Siberia.
Yield: 200-300 kilotons.
Great Britain
“Hurricane”: First nuclear test: October 3, 1952.
Location: Off Trimouille Island, Australia.
Yield: 25 kilotons.
Fissile material used: Plutonium-239.
Method of detonation: Implosion.
Method of production: Reactor.
Foreign Assistance: United States.
“Grapple Y”: Thought to be the first two-step thermonuclear test: April 28, 1958.
Location: Christmas Island.
Yield: 2 megatons.
Delivery Mechanism: Airdropped from a Valiant XD825 bomber.
France
“Gerboise Bleue”: First nuclear test: February 13, 1960.
Location: Reggane Proving Grounds, Algeria.
Yield: 60-70 kilotons.
Fissile material used: Plutonium-239.
Method of detonation: Implosion.
Method of production: Reactor.
“Canopus”: First thermonuclear test: August 24, 1968.
Location: Fangataufa Atoll.
Yield: 2.6 megatons.
Foreign assistance: Norway (heavy water to make tritium).
China
“596”: First nuclear test: October 16, 1964.
Location: Lop Nor.
Yield: 12.5-22 kilotons.
Fissile material used: Uranium-235.
Method of production: Gaseous diffusion.
Foreign assistance: Soviet Union.
First thermonuclear test: June 17, 1967.
Location: Lop Nor.
Yield: Approximately 3 megatons.
Delivery mechanism: Airdropped from a Hong 6 bomber.
Israel
Estimated date when first bomb was produced: Late 1966.
Fissile material: Plutonium.
Method of production: Dimona reactor imported from France and operated with heavy water supplied by Norway.
Probably conducted a 2-3 kiloton nuclear test on September 22, 1979 in the South Atlantic Ocean in cooperation with South Africa.
India
First nuclear test: May 18, 1974.
Location: Pokhran.
Yield: 2-15 kilotons.
Fissile material used: Plutonium-239.
Method of production: Cirus reactor supplied by Canada and operated with heavy water supplied by the United States.
Second nuclear test “Shakti 1”: May 11, 1998.
Location: Pokhran.
Yield: 10-15 kilotons.
Third nuclear test (claimed): May 13, 1998.
Yield: India claimed it tested two nuclear bombs, with a combined yield of 0.8 kilotons; however, there is no seismic evidence of any nuclear explosion.
South Africa
First device built: December 1982.
Total bombs built: Six.
Method of detonation: “Gun-type” device.
Fissile material used: Uranium-235.
Nuclear tests: None.
Dismantlement of the bomb program began in November 1989 and was completed in early September 1991, after which South Africa signed a comprehensive nuclear inspection agreement with the IAEA.
Pakistan
Estimated production of first bomb: Late 1987.
First nuclear test: May 28, 1998.
Location: Chagai Hills region.
Yield: 9-12 kilotons
Fissile material used: Uranium-235.
Method of production: Gas centrifuge technology smuggled from Europe.
Foreign assistance: China (bomb design), Germany (uranium processing equipment).
Second nuclear test: May 30, 1998.
Yield: 4-6 kilotons.
North Korea
First nuclear test: October 9, 2006.
Location: Near P’unggye-ri.
Yield: Less than 1 kiloton.
Fissile material used: Plutonium-239.
Method of production: Graphite moderated reactor at Yongbyon.
Second nuclear test: May 25, 2009.
Yield: 2 kilotons.
Fissile material used:
Undetermined (allegedly Plutonium-239).
Third nuclear test: February 12, 2013.
Yield: 3-7 kilotons.
Fissile material used: Undetermined.
Fourth nuclear test: January 6, 2016.
Yield: 6-10 kilotons.
Fissile material: Undetermined (allegedly included thermonuclear fuel).
Fifth nuclear test: September 9, 2016.
Yield: 10-20 kilotons.
Fissile Material: Undetermined